Introduction to the topic
Inheritance is one of the fundamental principles of biology, describing how traits and characteristics are passed from one generation to the next. The molecular basis of inheritance delves into the mechanisms by which genetic information, encoded in the DNA (Deoxyribonucleic Acid), is transmitted from parents to offspring, ensuring the continuity of life. This concept forms the cornerstone of genetics, a field that has revolutionized our understanding of biology, evolution, and medicine.
In the 19th century, Gregor Mendel’s experiments with pea plants laid the foundation for the principles of inheritance, which later became known as Mendelian genetics. However, the molecular basis of these principles remained unknown until the discovery of DNA in the 20th century. James Watson and Francis Crick, with their groundbreaking model of the DNA double helix, unveiled the structure of DNA in 1953, providing a tangible explanation for how genetic information is stored and replicated. This discovery marked a new era in biology, leading to the development of molecular genetics, a field that explores the molecular mechanisms behind genetic inheritance.
At the molecular level, inheritance involves the replication of DNA, the transcription of DNA into RNA (Ribonucleic Acid), and the translation of RNA into proteins. These proteins are responsible for the expression of traits, making DNA the blueprint of life. The study of the molecular basis of inheritance also encompasses various processes such as mutation, genetic recombination, and DNA repair, which contribute to genetic diversity and the evolution of species.
Understanding the molecular basis of inheritance is crucial not only for academic purposes but also for its practical applications. For instance, advances in genetic engineering, biotechnology, and personalized medicine are deeply rooted in our knowledge of how genes function at the molecular level. Moreover, this knowledge is pivotal in addressing challenges related to genetic disorders, agriculture, and environmental conservation.
This article aims to provide a detailed exploration of the molecular basis of inheritance, particularly in the context of the CBSE Standard XII Botany curriculum. It will cover the structure and function of DNA, the process of DNA replication, transcription, translation, and the regulation of gene expression. Additionally, it will discuss the significance of genetic mutations, genetic recombination, and the role of various enzymes in these processes.
Contents
Molecular Basis of Inheritance.
Regulation of Gene Expression.
Significance of the Central Dogma.
Genetic Engineering and Biotechnology.
Practical Applications of Molecular Genetics.
Molecular Basis of Inheritance Class 12: Important Questions and Answers.
Structure of DNA
The discovery of DNA’s structure was a monumental achievement in the field of biology. DNA, a polymer of nucleotides, consists of two long strands forming a double helix. Each nucleotide is composed of three components: a phosphate group, a deoxyribose sugar, and a nitrogenous base. There are four types of nitrogenous bases in DNA: adenine (A), thymine (T), cytosine (C), and guanine (G). The sequence of these bases encodes the genetic information.
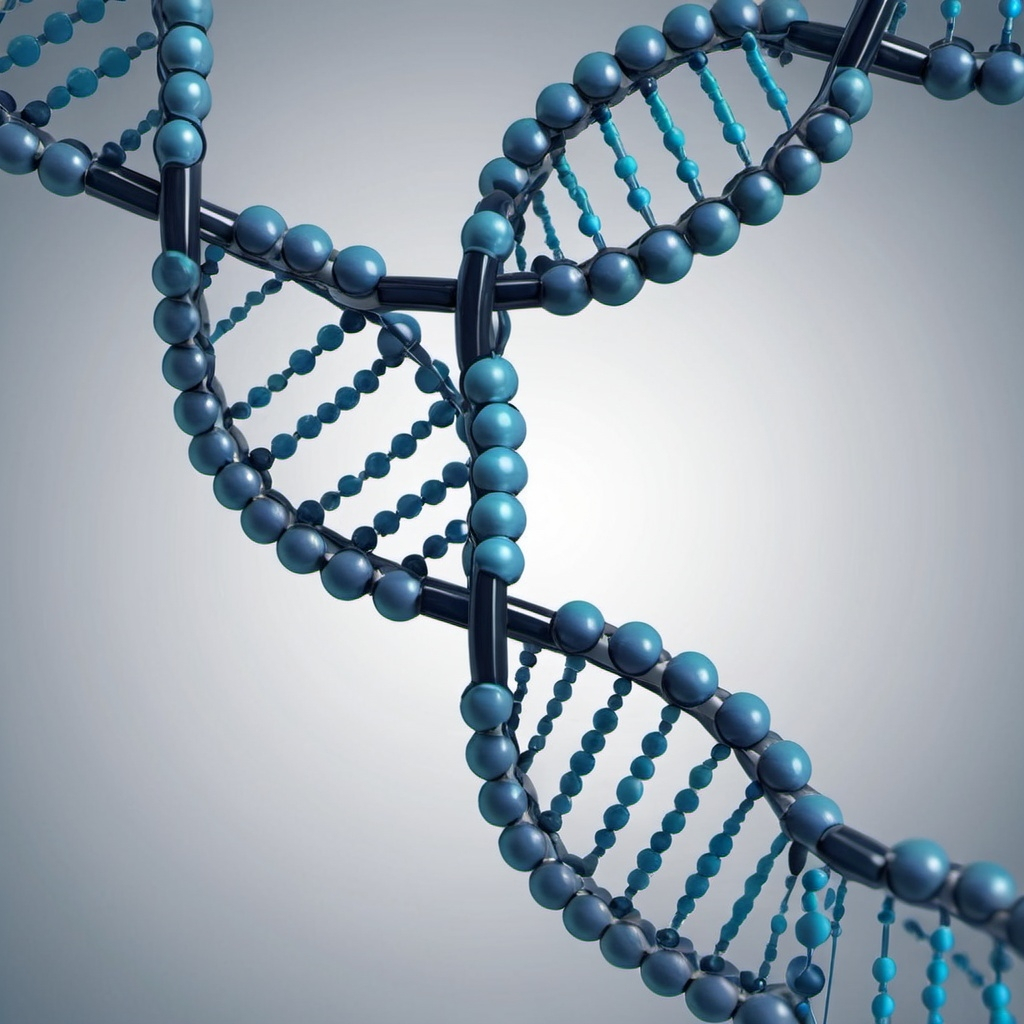
The two strands of DNA are antiparallel, meaning they run in opposite directions. The nitrogenous bases of one strand pair with those of the other strand through hydrogen bonds, following specific base-pairing rules: adenine pairs with thymine, and cytosine pairs with guanine. This complementary base pairing is crucial for the replication of DNA and the preservation of genetic information across generations.
“DNA’s double helix structure not only provides stability to the genetic material but also allows for its precise replication during cell division,” a fact that emphasizes the importance of understanding DNA structure in the study of inheritance.
DNA Replication
DNA replication is the process by which a cell duplicates its DNA, ensuring that each daughter cell receives an identical copy of the genetic material during cell division. This process is semi-conservative, meaning that each new DNA molecule consists of one original strand and one newly synthesized strand.
The replication process begins at specific sites on the DNA molecule called origins of replication. Here, enzymes known as helicases unwind the double helix, creating a replication fork. Single-strand binding proteins stabilize the unwound strands, preventing them from re-annealing. The enzyme primase synthesizes a short RNA primer, which serves as a starting point for DNA synthesis.
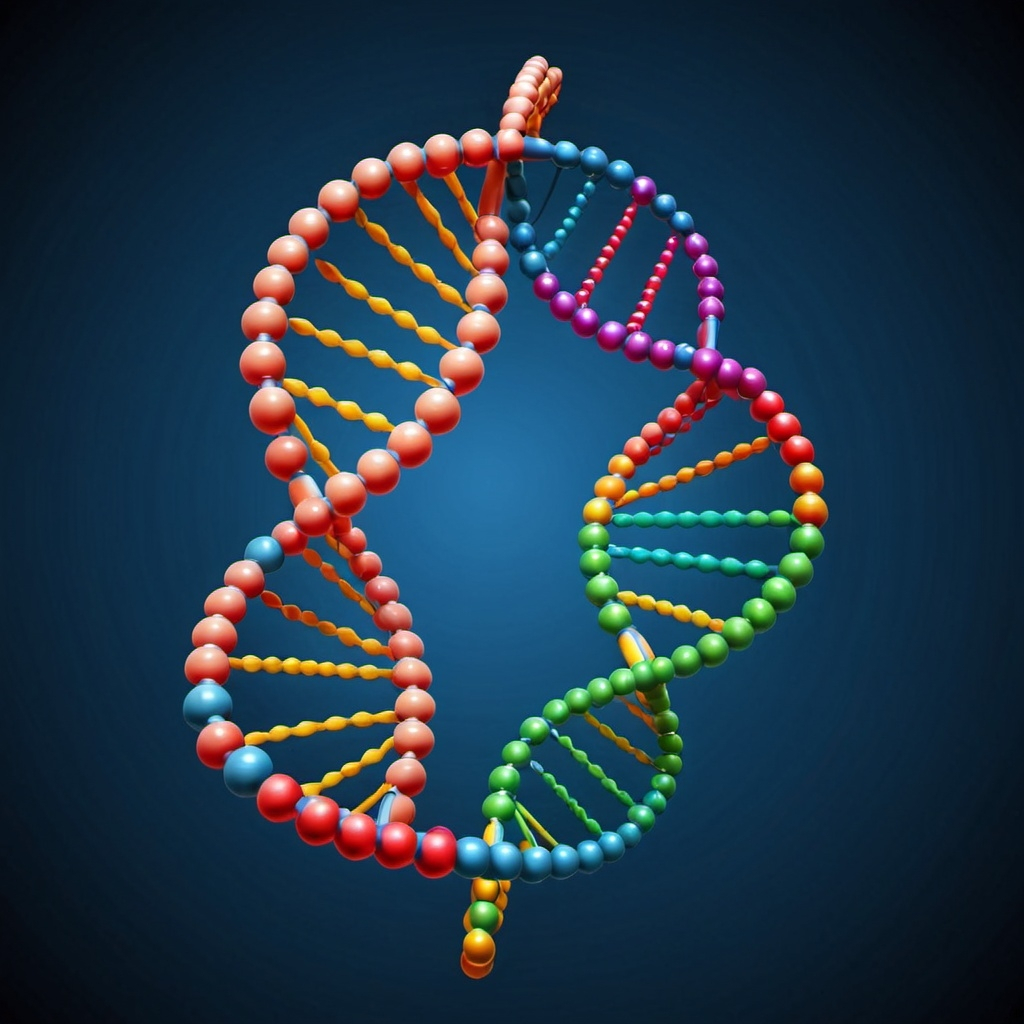
DNA polymerase, the enzyme responsible for adding nucleotides to the growing DNA strand, can only add nucleotides in the 5′ to 3′ direction. Therefore, DNA synthesis occurs continuously on the leading strand, which runs in the 3′ to 5′ direction relative to the replication fork. On the lagging strand, which runs in the 5′ to 3′ direction, DNA is synthesized in short fragments known as Okazaki fragments. These fragments are later joined together by the enzyme DNA ligase to form a continuous strand.
The accuracy of DNA replication is maintained by the proofreading function of DNA polymerase, which can detect and correct errors in the newly synthesized DNA. However, despite these mechanisms, errors can occasionally occur, leading to mutations.
“DNA replication is a highly regulated process, crucial for the maintenance of genetic integrity across generations,” a point that underscores the significance of replication in inheritance.
Transcription
Transcription is the process by which the information encoded in DNA is copied into messenger RNA (mRNA), which then carries this information to the ribosomes for protein synthesis. This process is the first step in the expression of genes.
Transcription begins when RNA polymerase binds to a specific region on the DNA called the promoter. The enzyme then unwinds the DNA and begins synthesizing a complementary RNA strand from the DNA template. The RNA strand grows in the 5′ to 3′ direction, with RNA polymerase adding ribonucleotides that are complementary to the DNA template strand.
In eukaryotic cells, the newly synthesized mRNA undergoes several modifications before it can be translated into a protein. These modifications include the addition of a 5′ cap, the addition of a poly-A tail at the 3′ end, and the removal of non-coding regions called introns through a process known as splicing. The resulting mature mRNA is then exported from the nucleus to the cytoplasm, where it is translated into a protein.
“The process of transcription is vital for the flow of genetic information from DNA to protein,” highlighting the central role of transcription in gene expression.
Translation
Translation is the process by which the information in mRNA is decoded to synthesize a protein. This process occurs in the ribosomes, which are the molecular machines responsible for protein synthesis.
The mRNA sequence is read in sets of three nucleotides, known as codons. Each codon specifies a particular amino acid, the building block of proteins. Transfer RNA (tRNA) molecules, which have an anticodon region complementary to the mRNA codon, bring the corresponding amino acid to the ribosome. The ribosome then links the amino acids together in the order specified by the mRNA sequence, forming a polypeptide chain.
Translation involves three main stages: initiation, elongation, and termination. During initiation, the ribosome assembles around the mRNA and the first tRNA. Elongation is the process of adding amino acids to the growing polypeptide chain, one by one, as the ribosome moves along the mRNA. Termination occurs when the ribosome reaches a stop codon on the mRNA, signaling the end of protein synthesis. The newly synthesized protein is then released and undergoes folding and modifications to become functional.
“Translation is the final step in the central dogma of molecular biology, where the genetic code is translated into a functional product,” emphasizing the importance of this process in gene expression.
Regulation of Gene Expression
Gene expression is tightly regulated to ensure that proteins are produced in the right amounts, at the right time, and in the right cells. Regulation occurs at multiple levels, including transcriptional, post-transcriptional, translational, and post-translational levels.
At the transcriptional level, gene expression can be regulated by the binding of transcription factors to specific DNA sequences near the promoter region. These factors can either enhance or repress the transcription of the gene. Epigenetic modifications, such as DNA methylation and histone modification, also play a crucial role in regulating gene expression by altering the accessibility of DNA to the transcriptional machinery.
Post-transcriptional regulation involves the processing of mRNA, including splicing, editing, and transport. Alternative splicing, for example, allows a single gene to produce multiple protein variants, increasing the diversity of the proteome.
Translational regulation controls the efficiency and timing of protein synthesis from mRNA, while post-translational regulation involves the modification of proteins after they are synthesized. These modifications can affect the stability, activity, and localization of proteins, thereby influencing their function.
“Regulation of gene expression is essential for the proper functioning of cells and organisms, enabling them to respond to internal and external signals,” highlighting the dynamic nature of gene regulation.
Genetic Mutations
Mutations are changes in the DNA sequence that can occur spontaneously or as a result of exposure to mutagens, such as radiation, chemicals, and viruses. Mutations can have various effects on an organism, ranging from neutral to beneficial or harmful, depending on their nature and location.
There are several types of mutations, including point mutations, insertions, deletions, and chromosomal rearrangements. Point mutations involve a change in a single nucleotide, which can lead to a change in the amino acid sequence of a protein. Insertions and deletions can cause a frameshift in the reading frame of the mRNA, potentially resulting in a completely different protein sequence. Chromosomal rearrangements involve large-scale changes in the structure of chromosomes, which can disrupt multiple genes.
Some mutations are passed on to the next generation, contributing to genetic diversity and evolution. However, mutations can also lead to genetic disorders and diseases. For example, sickle cell anemia is caused by a point mutation in the gene encoding the β-globin protein, leading to the production of abnormal hemoglobin.
“Mutations are a source of genetic variation, but they can also have significant consequences for an organism’s health and survival,” emphasizing the dual role of mutations in evolution and disease.
Genetic Recombination
Genetic recombination is a process that occurs during meiosis, the type of cell division that produces gametes (sperm and eggs) in sexually reproducing organisms. Recombination involves the exchange of genetic material between homologous chromosomes, leading to the creation of new combinations of genes in the offspring. This process increases genetic diversity within a population, which is essential for evolution and adaptation to changing environments.
During prophase I of meiosis, homologous chromosomes pair up and exchange segments of DNA through a process called crossing over. The points of exchange are known as chiasmata. The result is that the chromosomes in the gametes have a mixture of alleles from both parents, contributing to the genetic uniqueness of each individual.
Recombination is not only important for generating genetic diversity but also plays a crucial role in DNA repair. For example, if a chromosome sustains damage, recombination with a homologous chromosome can help repair the break by providing an undamaged template.
“Genetic recombination is a vital mechanism for increasing genetic variability, which is the raw material for natural selection,” highlighting its importance in evolution.
DNA Repair Mechanisms
DNA is constantly subjected to damage from various sources, including UV radiation, chemical agents, and errors during replication. To maintain the integrity of the genetic material, cells have evolved several DNA repair mechanisms that correct damage and prevent mutations from being passed on to the next generation.
One of the primary DNA repair mechanisms is base excision repair (BER), which corrects small, non-helix-distorting lesions in the DNA. This process involves the removal of damaged bases by specific enzymes called DNA glycosylases, followed by the excision of the sugar-phosphate backbone at the site of damage. DNA polymerase then fills in the gap with the correct nucleotide, and DNA ligase seals the strand.
Another important repair mechanism is nucleotide excision repair (NER), which is responsible for repairing bulky, helix-distorting lesions, such as those caused by UV-induced thymine dimers. NER involves the removal of a short segment of the damaged strand, followed by synthesis of the missing portion using the undamaged strand as a template.
“Mismatch repair (MMR) is a crucial mechanism that corrects errors introduced during DNA replication, ensuring the fidelity of the genetic code,” emphasizing the importance of DNA repair in maintaining genetic stability.
Defects in DNA repair mechanisms can lead to an accumulation of mutations, which can result in genetic disorders and contribute to the development of cancer. For example, mutations in the BRCA1 and BRCA2 genes, which are involved in homologous recombination repair, are associated with an increased risk of breast and ovarian cancer.
Significance of the Central Dogma
The central dogma of molecular biology describes the flow of genetic information within a biological system: DNA → RNA → Protein. This concept, first proposed by Francis Crick in 1958, outlines the processes of transcription and translation that lead to the expression of genes as functional proteins.
The central dogma is significant because it provides a framework for understanding how genetic information is transferred and expressed in living organisms. It also highlights the importance of each step in the process, from the accurate replication of DNA to the precise synthesis of proteins that carry out cellular functions.
“Understanding the central dogma is fundamental to molecular biology, as it explains how genetic information is stored, transmitted, and utilized within cells,” emphasizing the concept’s central role in genetics.
In recent years, the central dogma has been expanded to include the roles of non-coding RNAs, such as microRNAs and long non-coding RNAs, which regulate gene expression at various levels. These discoveries have added complexity to our understanding of gene regulation and have opened new avenues for research in molecular biology and medicine.
Genetic Engineering and Biotechnology
Advances in our understanding of the molecular basis of inheritance have paved the way for the development of genetic engineering and biotechnology. Genetic engineering involves the manipulation of an organism’s DNA to introduce new traits or modify existing ones. This technology has a wide range of applications in medicine, agriculture, and industry.
One of the most well-known applications of genetic engineering is the production of genetically modified organisms (GMOs). In agriculture, GMOs have been developed to increase crop yields, improve nutritional content, and confer resistance to pests and diseases. For example, Bt cotton, a genetically modified crop, produces a protein from the bacterium Bacillus thuringiensis that is toxic to certain insects, reducing the need for chemical pesticides.
In medicine, genetic engineering has led to the development of gene therapy, a technique that involves the insertion of healthy genes into an individual’s cells to treat genetic disorders. For example, gene therapy has been used to treat severe combined immunodeficiency (SCID) by introducing a functional copy of the defective gene into the patient’s bone marrow cells.
“CRISPR-Cas9, a revolutionary gene-editing tool, allows for precise modifications to the DNA sequence, offering potential cures for genetic diseases and advancements in biotechnology,” underscoring the impact of modern genetic engineering techniques.
Biotechnology also includes the production of recombinant proteins, such as insulin, which is used to treat diabetes. By inserting the human insulin gene into bacteria, scientists can produce large quantities of insulin for therapeutic use.
Practical Applications of Molecular Genetics
The principles of molecular genetics have numerous practical applications in various fields, including medicine, agriculture, and forensic science. Understanding the molecular basis of inheritance has enabled significant advancements in these areas, improving the quality of life and addressing global challenges.
In medicine, molecular genetics is used in the diagnosis and treatment of genetic disorders. Techniques such as polymerase chain reaction (PCR) and DNA sequencing allow for the identification of genetic mutations associated with diseases, enabling early diagnosis and personalized treatment plans. For example, BRCA gene testing can identify individuals at high risk for breast and ovarian cancer, allowing for preventive measures or targeted therapies.
In agriculture, molecular genetics has led to the development of crops with enhanced traits, such as drought tolerance, pest resistance, and improved nutritional content. These genetically modified crops have the potential to increase food production and address food security issues, particularly in regions with challenging growing conditions.
Forensic science has also benefited from molecular genetics, with DNA profiling becoming a standard tool for identifying individuals in criminal investigations. The analysis of genetic material from crime scenes can link suspects to evidence, exonerate the innocent, and provide critical leads in solving cases.
“DNA fingerprinting, a technique based on genetic variations, has revolutionized forensic science by providing a reliable method for individual identification,” highlighting the role of molecular genetics in criminal justice.
Ethical Considerations
The advances in molecular genetics and genetic engineering have raised several ethical considerations that must be addressed as these technologies continue to evolve. Issues such as genetic privacy, the potential for genetic discrimination, and the implications of gene editing on future generations are at the forefront of ethical discussions.
One of the major concerns is the potential for genetic information to be used in ways that could harm individuals or groups. For example, the misuse of genetic data by employers or insurance companies could lead to discrimination based on an individual’s genetic predisposition to certain diseases.
The ethical implications of gene editing, particularly germline editing (which affects future generations), are also a topic of debate. While gene editing has the potential to eliminate genetic disorders, it also raises concerns about unintended consequences, such as off-target effects and the potential for “designer babies,” where genetic traits could be selected based on personal preferences rather than medical necessity.
“Ethical considerations in molecular genetics are critical to ensuring that the benefits of these technologies are realized while minimizing potential risks and respecting individual rights,” emphasizing the importance of ethical oversight in the field.
Molecular Basis of Inheritance Class 12: Important Questions and Answers
1. What is the structure of DNA?
Answer:
DNA, or deoxyribonucleic acid, is a double-helix structure composed of two strands running in opposite directions. The backbone of each strand is made up of alternating sugar (deoxyribose) and phosphate groups. The nitrogenous bases, which include adenine (A), thymine (T), cytosine (C), and guanine (G), are attached to the sugar molecules and form the rungs of the helix. Adenine pairs with thymine through two hydrogen bonds, while cytosine pairs with guanine through three hydrogen bonds, maintaining a consistent width of the DNA helix.
2. Describe the process of DNA replication.
Answer:
DNA replication is the process by which a DNA molecule makes a copy of itself. This process occurs during the S phase of the cell cycle. It involves the following steps:
- Initiation: The DNA double helix unwinds at specific regions called origins of replication, forming a replication fork.
- Elongation: DNA polymerase synthesizes the new DNA strand by adding complementary nucleotides to the template strand. The leading strand is synthesized continuously, while the lagging strand is synthesized in short fragments called Okazaki fragments.
- Termination: Once the entire molecule is replicated, the replication process concludes, and the two DNA molecules separate.
The newly formed DNA molecules consist of one parental strand and one newly synthesized strand, making the process semi-conservative.
3. Explain the central dogma of molecular biology.
Answer:
The central dogma of molecular biology describes the flow of genetic information within a cell. It states that information in cells flows from DNA to RNA to proteins. This involves two key processes:
- Transcription: DNA is transcribed into messenger RNA (mRNA) in the nucleus. The mRNA carries the genetic code from the DNA to the ribosomes in the cytoplasm.
- Translation: The mRNA is translated into a specific sequence of amino acids, leading to the synthesis of proteins. This process takes place in the ribosomes.
The central dogma is fundamental to understanding how genes control cellular functions and how genetic information is expressed.
4. What are mutations, and how do they occur?
Answer:
Mutations are changes in the nucleotide sequence of DNA. They can occur spontaneously during DNA replication or be induced by external factors such as radiation, chemicals, or viruses. Mutations can be of several types, including:
- Point mutations: A change in a single nucleotide base, which can result in a silent, missense, or nonsense mutation.
- Frameshift mutations: Insertions or deletions of nucleotides that alter the reading frame of the genetic code.
- Chromosomal mutations: Large-scale changes involving sections of chromosomes, such as deletions, duplications, inversions, or translocations.
Mutations can lead to various genetic disorders if they affect genes essential for normal cellular function.
5. Describe the process of transcription in detail.
Answer:
Transcription is the process of synthesizing RNA from a DNA template. It occurs in the nucleus and involves the following steps:
- Initiation: RNA polymerase binds to the promoter region of the gene, which signals the start of transcription.
- Elongation: RNA polymerase moves along the DNA template strand, adding complementary RNA nucleotides (adenine pairs with uracil instead of thymine in RNA).
- Termination: The process continues until RNA polymerase reaches a terminator sequence, signaling the end of transcription. The newly synthesized mRNA strand detaches from the DNA template.
The mRNA then undergoes processing, including the addition of a 5′ cap, a poly-A tail, and splicing to remove introns before being transported to the cytoplasm for translation.
6. What is the role of tRNA in translation?
Answer:
Transfer RNA (tRNA) plays a crucial role in translation, the process of synthesizing proteins from mRNA. Each tRNA molecule has an anticodon, a set of three nucleotides that are complementary to a specific codon on the mRNA strand. At the other end of the tRNA, a specific amino acid is attached.
During translation, tRNA molecules carry amino acids to the ribosome, where the anticodon pairs with the corresponding mRNA codon. This ensures that the amino acids are added in the correct sequence to form a polypeptide chain, which eventually folds into a functional protein.
7. What is the significance of the genetic code being universal?
Answer:
The genetic code is considered universal because it is nearly identical in all living organisms. This means that a particular codon in mRNA specifies the same amino acid in all species, from bacteria to humans. The universality of the genetic code has several implications:
- It suggests a common evolutionary origin for all life forms.
- It allows for the transfer of genes between different organisms, enabling genetic engineering and biotechnology applications.
- It ensures that the fundamental processes of transcription and translation are consistent across species, making it easier to study gene function and regulation.
8. Explain the concept of genetic recombination.
Answer:
Genetic recombination is the process by which genetic material is exchanged between homologous chromosomes during meiosis. This exchange occurs through a mechanism called crossing over, where segments of DNA are swapped between paired chromosomes. Recombination results in new combinations of alleles, contributing to genetic diversity in offspring.
This process is crucial for evolution, as it generates variability in populations, providing raw material for natural selection. Genetic recombination also plays a role in DNA repair, ensuring the integrity of genetic material is maintained.
9. What are operons, and how do they regulate gene expression in prokaryotes?
Answer:
Operons are clusters of genes in prokaryotes that are transcribed together as a single mRNA strand. They consist of structural genes, a promoter, an operator, and regulatory genes. The operon model, first proposed by François Jacob and Jacques Monod, explains how gene expression is regulated in bacteria.
One of the best-known operons is the lac operon in Escherichia coli. It controls the metabolism of lactose. When lactose is present, it binds to the repressor protein, preventing it from blocking the operator. This allows RNA polymerase to transcribe the structural genes needed to metabolize lactose. When lactose is absent, the repressor binds to the operator, preventing transcription.
This system allows bacteria to conserve energy by only expressing genes when their products are needed.
10. What is the role of epigenetics in gene regulation?
Answer:
Epigenetics refers to changes in gene expression that do not involve alterations to the DNA sequence itself. These changes are often influenced by environmental factors and can be passed on to subsequent generations. Epigenetic regulation occurs through mechanisms such as DNA methylation, histone modification, and non-coding RNAs.
- DNA methylation involves the addition of a methyl group to the DNA, typically at cytosine bases, leading to the repression of gene expression.
- Histone modification involves changes to the proteins around which DNA is wound, affecting the accessibility of genes to the transcription machinery.
- Non-coding RNAs can regulate gene expression by interfering with mRNA or modifying chromatin structure.
Epigenetic changes play a crucial role in development, differentiation, and disease, as they can activate or silence genes in response to internal and external signals.
11. How does DNA fingerprinting work?
Answer:
DNA fingerprinting is a technique used to identify individuals based on their unique genetic makeup. It involves analyzing specific regions of the DNA that vary greatly among individuals, such as short tandem repeats (STRs).
The process typically involves the following steps:
- Extraction: DNA is extracted from biological samples such as blood, hair, or saliva.
- Amplification: The STR regions are amplified using polymerase chain reaction (PCR) to generate enough DNA for analysis.
- Separation: The amplified DNA fragments are separated by size using gel electrophoresis or capillary electrophoresis.
- Comparison: The pattern of STRs is compared between samples to determine a match.
DNA fingerprinting is widely used in forensic science for identifying suspects, establishing paternity, and identifying victims of disasters.
12. What is the significance of the Human Genome Project?
Answer:
The Human Genome Project (HGP) was an international research effort to map and sequence the entire human genome, which consists of approximately 3 billion base pairs of DNA. Completed in 2003, the HGP has had a profound impact on biology and medicine.
The significance of the HGP includes:
- Understanding genetic diseases: The project has identified genes associated with various genetic disorders, paving the way for diagnostic tests, treatments, and potential cures.
- Advancing personalized medicine: Knowledge of the human genome has enabled the development of personalized treatments based on an individual’s genetic makeup.
- Enhancing evolutionary studies: The HGP has provided insights into human evolution and the genetic relationships between different species.
- Fostering biotechnological innovations: The data generated by the HGP has been used in various biotechnological applications, including drug development and gene therapy.
The HGP has revolutionized our understanding of genetics and continues to influence research and healthcare.
13. Explain the role of RNA in the process of protein synthesis.
Answer:
RNA plays several critical roles in the process of protein synthesis, which involves the translation of genetic information from mRNA into a polypeptide chain. The three main types of RNA involved are:
- Messenger RNA (mRNA): mRNA carries the genetic code transcribed from DNA to the ribosomes, where protein synthesis occurs. The sequence of codons in mRNA dictates the order in which amino acids are added to the growing polypeptide chain.
- Transfer RNA (tRNA): tRNA is responsible for bringing the correct amino acids to the ribosome during translation. Each tRNA molecule has an anticodon that pairs with the corresponding codon on the mRNA strand, ensuring that amino acids are added in the proper sequence.
- Ribosomal RNA (rRNA): rRNA is a component of ribosomes, the cellular structures where protein synthesis takes place. rRNA helps to catalyze the formation of peptide bonds between amino acids, facilitating the assembly of the polypeptide chain.
Together, these three types of RNA work in concert to translate the genetic code into functional proteins, which perform a wide range of essential roles within the cell.
14. What are the key differences between prokaryotic and eukaryotic transcription?
Answer:
Transcription in prokaryotes and eukaryotes shares many similarities, but there are also significant differences:
- Location: In prokaryotes, transcription occurs in the cytoplasm since they lack a nucleus. In contrast, eukaryotic transcription occurs in the nucleus.
- RNA Polymerase: Prokaryotes typically have a single type of RNA polymerase that synthesizes all types of RNA. Eukaryotes, however, have three different RNA polymerases: RNA polymerase I (for rRNA), RNA polymerase II (for mRNA), and RNA polymerase III (for tRNA and other small RNAs).
- Post-Transcriptional Modifications: Eukaryotic mRNA undergoes significant processing after transcription, including the addition of a 5′ cap, poly-A tail, and splicing to remove introns. In prokaryotes, mRNA does not undergo such extensive modifications and is often translated directly as it is being transcribed.
- Regulation: In prokaryotes, gene regulation often involves operons, where multiple genes are transcribed together as a single mRNA. Eukaryotic gene regulation is more complex, involving various regulatory elements such as enhancers, silencers, and transcription factors.
These differences reflect the greater complexity and compartmentalization of eukaryotic cells compared to prokaryotic cells.
15. How do ribosomes function in protein synthesis?
Answer:
Ribosomes are the molecular machines that facilitate the translation of mRNA into a polypeptide chain, ultimately leading to protein synthesis. The process occurs in the following steps:
- Initiation: The small ribosomal subunit binds to the mRNA at the start codon (AUG). A tRNA molecule carrying methionine, the first amino acid, binds to the start codon. The large ribosomal subunit then assembles with the small subunit, forming a functional ribosome.
- Elongation: The ribosome moves along the mRNA strand, reading each codon and facilitating the binding of the corresponding tRNA carrying the appropriate amino acid. As each amino acid is added, it forms a peptide bond with the preceding amino acid, elongating the polypeptide chain.
- Termination: When the ribosome reaches a stop codon on the mRNA (UAA, UAG, or UGA), translation ends. The newly synthesized polypeptide chain is released, and the ribosomal subunits dissociate.
Ribosomes ensure that the genetic code carried by mRNA is accurately translated into a sequence of amino acids, forming the specific proteins required for cellular function.
16. What is the role of non-coding RNA in gene regulation?
Answer:
Non-coding RNAs (ncRNAs) are RNA molecules that are not translated into proteins but play critical roles in regulating gene expression. Some key types of non-coding RNA include:
- MicroRNAs (miRNAs): miRNAs are small, single-stranded RNA molecules that bind to complementary sequences on target mRNA molecules, typically leading to the repression of translation or the degradation of the mRNA.
- Small interfering RNAs (siRNAs): siRNAs are involved in the RNA interference (RNAi) pathway, where they guide the degradation of complementary mRNA, thereby silencing specific genes.
- Long non-coding RNAs (lncRNAs): lncRNAs are longer RNA molecules that can regulate gene expression at various levels, including chromatin remodeling, transcriptional regulation, and post-transcriptional processing.
- Ribosomal RNA (rRNA) and Transfer RNA (tRNA): While these RNAs are involved in the fundamental process of translation, they are also considered non-coding because they do not code for proteins.
Non-coding RNAs are essential in fine-tuning gene expression and play a role in development, differentiation, and the maintenance of cellular homeostasis.
17. Explain the concept of alternative splicing.
Answer:
Alternative splicing is a process by which a single gene can produce multiple mRNA variants, leading to the production of different proteins from the same genetic sequence. During the processing of precursor mRNA (pre-mRNA), certain exons (coding regions) may be included or excluded, resulting in different combinations of exons in the mature mRNA.
This process allows for the generation of protein diversity without the need for additional genes. Alternative splicing plays a crucial role in development and tissue specificity, as well as in the regulation of gene expression in response to environmental cues.
18. What is gene cloning, and how is it performed?
Answer:
Gene cloning is the process of making multiple identical copies of a specific gene or DNA sequence. It involves the following steps:
- Isolation of the DNA Sequence: The gene of interest is isolated from the organism’s genome using restriction enzymes that cut DNA at specific sequences.
- Insertion into a Vector: The isolated gene is inserted into a cloning vector, typically a plasmid (a small, circular piece of DNA found in bacteria). The plasmid also contains a selectable marker, such as antibiotic resistance, to identify cells that have taken up the plasmid.
- Transformation: The recombinant plasmid is introduced into a host cell, usually a bacterium, through a process called transformation.
- Selection: The transformed cells are grown on a selective medium containing an antibiotic. Only cells that have successfully taken up the plasmid (and therefore have antibiotic resistance) will survive.
- Replication: As the bacteria divide, they replicate the plasmid along with their own DNA, producing many copies of the gene of interest.
Gene cloning is a fundamental technique in molecular biology and has numerous applications in research, medicine, and biotechnology.
19. What are the ethical considerations related to genetic engineering?
Answer:
Genetic engineering, while offering significant potential for advances in medicine, agriculture, and other fields, also raises important ethical considerations:
- Human Genetic Modification: The possibility of editing human genes, particularly in embryos, raises concerns about the long-term consequences, including the potential for unintended effects, the ethical implications of “designer babies,” and issues of consent.
- Environmental Impact: The release of genetically modified organisms (GMOs) into the environment could have unforeseen ecological consequences, such as the disruption of local ecosystems or the spread of modified genes to wild populations.
- Equity and Access: Advances in genetic engineering could exacerbate social inequalities if access to these technologies is limited to certain groups, leading to disparities in health, agriculture, and economic opportunities.
- Biodiversity: The widespread use of genetically engineered crops could reduce genetic diversity in agriculture, making crops more vulnerable to pests, diseases, and changing environmental conditions.
- Animal Welfare: Genetic engineering of animals, particularly for research or agricultural purposes, raises questions about the ethical treatment of animals and the potential for suffering.
Ethical considerations must be carefully weighed in the development and application of genetic engineering technologies to ensure they are used responsibly and for the benefit of all.
Conclusion
The molecular basis of inheritance is a cornerstone of modern biology, providing a comprehensive understanding of how genetic information is transmitted, expressed, and regulated in living organisms. From the discovery of DNA’s structure to the development of genetic engineering techniques, our knowledge of molecular genetics has expanded dramatically, leading to significant advancements in medicine, agriculture, and biotechnology.
The study of the molecular basis of inheritance is not only essential for academic purposes but also has practical implications that impact our daily lives. Understanding how genes function at the molecular level enables the development of new therapies for genetic disorders, the creation of genetically modified crops to address food security, and the use of DNA profiling in forensic science.
However, as we continue to explore the possibilities offered by molecular genetics, it is crucial to address the ethical considerations associated with these technologies. Ensuring that genetic information is used responsibly and that the potential risks of genetic engineering are carefully managed is essential for the continued advancement of the field.
“Knowledge of the molecular basis of inheritance is key to unlocking the potential of genetic technologies, offering solutions to some of the most pressing challenges facing humanity today,” underscoring the importance of ongoing research and ethical considerations in the field of molecular genetics.
This comprehensive exploration of the molecular basis of inheritance is aligned with the CBSE Standard XII Botany curriculum. It provides a detailed understanding of the key concepts, including DNA structure, replication, transcription, translation, gene regulation, and the practical applications of molecular genetics.
Disclaimer
Although these questions and answers provide a comprehensive overview of the key concepts covered in the “Molecular Basis of Inheritance” chapter in the CBSE Class 12 Biology curriculum. Please always cross compare and check the information with your text books.
Understanding these concepts is essential for students to grasp the complexities of genetic information and its role in the functioning of living organisms. By mastering these topics, students will be well-prepared to excel in their exams and further studies in the field of biology.
Best Reads
Your article helped me a lot, is there any more related content? Thanks!